Accelerating the drug-discovery process: new tools and technologies available to medicinal chemists


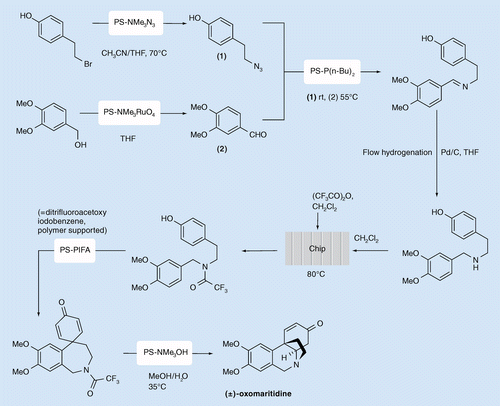
rt: Room temperature.
One of the main issues that the pharmaceutical industry has had to face in recent years is the reduced efficiency of the drug-discovery process, despite the impressive amounts of money devoted yearly to R&D (>US$50 billion in 2007). The number of new chemical entities that survive the clinical phases and get onto the market is decreasing: most drug candidates fail in advanced development (especially Phases I or II), after which a huge amount of money has already been spent on their discovery. For this reason, the pharmaceutical industry has been forced to rethink the drug-discovery process with the objective of identifying earlier drug candidates that have the highest chances of becoming successful drugs. Since many drug candidates fail in clinical trials due to liabilities associated with poor pharmacokinetics, low efficacy and high toxicity, current drug-discovery strategies aim to identify these liabilities early on, to avoid late-stage failures. This means that during the hit validation and lead optimization phases, compounds are optimized not only on the basis of their activity or selectivity for the molecular target of interest, but also for their solubility, drug likeness, pharmacokinetic profile and predicted toxicity (the so-called multiparametric lead optimization approach). It is the compound with the best balance of all these properties that is selected for development, rather than the most active compound.
This new strategy in the drug-discovery process has also impacted, to some extent, on the approach to medicinal chemistry. At the end of 1990s, robotized techniques and, in particular, high-throughput screening (HTS) were the main approaches used to find new hit compounds. One of the main objectives of the medicinal chemist was to synthesize a sufficiently large number of compounds to fill the HTS machinery. Combinatorial chemistry was the chemical answer to HTS and was initially perceived by many opinion leaders as a sort of ‘holy grail’ due to its supposed capacity to overcome the bottleneck, by speeding up the drug-discovery process with huge compound libraries being synthesized, often on the solid phase. Each compound was synthesized in low or very low amounts, just enough to test its primary activity but not to profile it further. To synthesize very big libraries, we often saw a significant compromise in terms of the ‘quality’ of the compounds (e.g., low purity and small diversity) and easily-accessible chemical scaffolds were privileged. Low-quality hits were often identified and these were then optimized to low-quality leads that did not translate into increased productivity of development candidates. In the new multiparametric lead-optimization approach, most compounds are profiled from the beginning using a number of different tests, ranging from activity at the desired molecular target and selectivity to microsomal stability, interaction with P450 isoenzymes, permeability, plasma protein binding and solubility. This results in a higher amount of each compound being synthesized from the beginning. Moreover, greater attention is being paid to the drug-likeness of the chemical structures being designed and the medicinal chemist is asked to synthesize more challenging scaffolds, if these are predicted to be more drug-like. In the last 5 years, huge compound libraries have disappeared, solid-phase chemistry has been rarely used and new solution-phase technologies have been developed to better assist the medicinal chemist in approaching these new tasks. Among the most significant technical improvements, the greatest impact has been cheated by polymer-assisted solution-phase synthesis, microwave synthesis and, more recently, flow chemistry.
Polymer-assisted solution-phase synthesis
Although the era of combinatorial chemistry and huge combinatorial libraries has come to an end, the possibility of synthesizing small libraries of compounds (24–500 compounds) is still a good option for speeding up the lead-optimization phase, particularly if these compounds can be synthesized in fairly high quantities (30–50 mg). This can be easily obtained by a solution-phase parallel-synthesis approach and specific equipment has been developed to help the chemist in this regard. The main challenges when working in parallel and in solution are associated with the work-up and purification phases. Polymer-assisted solution-phase synthesis (PASP) has been developed to address these issues. PASP utilizes a polymer to speed up the work-up and purification phases, which will consist of simple filtrations and washings. This polymer is called a scavenger when it is used to remove unreacted reagents or by-products from the reaction mixture; it is called a polymer-supported reagent when it is attached to the reactive species that transform the starting material into the desired product. In this case, the reagent can be used in strong excess to drive the reaction to completion, since it will be easy to remove the unreacted reagent by simple filtration. Moreover, solid-supported reagents are less toxic and easier to manage than the corresponding ‘nonpolymer-bound’ reagents, improving their industrial acceptability and safety profile. The main drawbacks are their lower reactivity (due to the diffusion kinetics of the solution species into the solid support) and their higher costs, which can become an issue particularly when scale-up is considered.
Polymer-assisted solution-phase synthesis has been used not only to synthesize libraries of compounds in solution, but also to prepare natural compounds utilizing multistep synthetic pathways. The first example of the total synthesis of natural products using a sequence of polymer-supported reagents is shown in Figure 1, namely the total synthesis of the racemic form of oxomaritidine and epimaritidine [1]. All synthetic steps were performed using solid-supported reagents and these steps range from oxidations and reductive alkylations to oxidative cyclizations and reductions. The products were obtained in six to seven synthetic steps, in excellent yields and at high levels of purity without the use of any chromatographic purification.
The commercial availability of a large variety of reagents in the supported form makes the use of PASP particularly versatile and attractive to organic chemists. Moreover, supported reagents can also be employed in combination with other emerging technologies, such as microwave heating and flow chemistry, thus further enhancing the efficiency of the entire synthetic process.
Microwave-assisted organic synthesis
Another way to speed up the hit-validation and lead-optimization phases is to decrease the time required to perform chemical reactions. One of the main advantages of microwave heating, when compared with traditional heating, is a dramatic reduction of reaction times, typically from days or hours to minutes or even seconds. In addition, microwave synthesis allows the discovery of novel reaction pathways, expanding the available ‘chemical space’ and allowing the synthesis of more complex structures.
Microwave energy started being applied in heating foodstuffs in the 1940s and found application in the chemical industry in the 1950s. Only since the mid-1980s has it been utilized in organic synthesis. In 1986, two different groups (those of Richard Gedye [2] and Giguere [3]) published the first reports on the use of microwave chemistry to accelerate organic transformations. In those days, domestic microwave ovens were utilized, causing safety issues and reproducibility problems due to poor homogeneity in the microwave field. Since 2000, the first chemistry-dedicated microwave equipment started being produced, allowing a rapid expansion of microwave-assisted organic synthesis not only in the academic environment, but also in the pharmaceutical industry. This equipment ensures the homogeneity of the microwave field (and thus the reproducibility of the reactions) and allows temperature and pressure monitoring within the reaction vessel. Explosion-proof cavities allow safe application of microwave heating in closed vessels, where solvents are heated well above their boiling points.
Microwave chemistry is mainly based on the efficient heating of materials by microwave dielectric heating effect. Dipoles of solvents or reagents try to align themselves with the oscillating electric component of microwaves and this continuous reorientation produces molecular friction and dielectric loss that generate energy in the form of heat. This effect is known as dipolar polarization. A second major heating mechanism is known as ionic conduction. Ions in a reaction mixture oscillate under the influence of the microwave field and collide with other molecules creating heat. Microwave irradiation produces a more efficient internal heating in comparison to the traditional external heating source, such as the oil bath, which transfers heat by conductance. In this way, the temperature is more uniform and formation of by-products is reduced.
In summary, many chemical reactions (particularly those that need a high reaction temperature) gain the following advantages from the use of microwave irradiation:
▪ Reduction of reaction times is obtained by the very fast ‘in-core’ heating of reaction mixtures caused by microwaves and by the fact that reactions are often conducted at higher temperatures, in sealed vessels;
▪ Higher yields and a cleaner reaction profile, due to the fact that reactions are conducted under pressure and the heating is uniform;
▪ The possibility of using lower boiling point solvents under pressure (in sealed vessels) in a safe environment, since modern microwaves have an explosion-proof cavity;
▪ Reproducible experimental conditions by accurate on-line control of temperature and pressure profile;
▪ Specific heating of strongly microwave-absorbing metal catalysts.
As an example, the synthesis of 3-methyl-2-phenylquinoline-4-carboxylic acid via Pfitzinger reaction is shown in Figure 2[4]. It can be seen how the use of microwave heating in sealed vessel allows a reduction of the reaction time from 48 to 2 h and an increase in the yield from 44 to 97%. Using the same procedure, a small library of 3-methyl-2-phenylquinoline-4-carboxylic acids, additionally substituted at positions 6 and 7 of the quinoline ring, was prepared in our laboratories [4].
Flow chemistry
While chemical reactions in the medicinal chemistry laboratories are still usually performed utilizing essentially the same glassware that was used more than 100 years ago, the need to find a technology that could be very flexible given the different quantities of material required within the different phases of the drug-discovery process (from a few milligrams in the lead-optimization phase, to 50–100 g when a candidate is selected for toxicological studies, to 0.5–5 kg when Phase I is started) fostered the development of flow chemistry. In flow chemistry, a chemical reaction is performed by continuously pumping a flowing stream of reagents in a network of interconnecting tubes; where the tubes join, the reagents come into contact and the reaction takes place. Clearly, the amount of material synthesized will depend on the time the flow system is allowed to run; increasing this time, without changing the other reaction conditions, will simply result in the production of higher quantities of material (scaling out). This situation is completely different from batch chemistry, where scaling up usually implies a new optimization of the reaction conditions.
When describing flow reactors, there are two scales that are of interest to the medicinal chemist: meso- and micro-reactors. The former have a typical diameter of tubes ranging from 1 to 10 mm and the latter have a diameter of less than 1 mm. Both types of reactors have been produced in a range of different materials, including glass, ceramics, polymers and metals.
In addition to the facile transfer among scales, the main advantages of flow chemistry include extremely rapid mixing and heat transfer and the elimination of dosing time, resulting in constant mixture composition. Moreover, toxic, explosive or unstable intermediates are reacted in situ, without accumulation, thus increasing the safety profile and decreasing by-product formation. Additional advantages of flow chemistry are connected to the possibility of coupling this technique to microwave heating or to PASP, by simply pumping the reaction mixture through cartridges containing polymer-supported reagents or scavengers. Individual reactions can be linked into multistep pathways assembling a line of flow reactors, providing that solvent switching is not required, thus creating rapid routes to complex structures. Online monitoring of product formation by LC–MS analysis is also feasible and the possibility to couple flow synthesis to direct biological evaluation in a single platform has been demonstrated [5].
One main issue associated with flow chemistry is that reagents and products must be soluble in the solvent flow, since flow systems are incompatible with precipitating products, especially when microfluidic systems are employed. As an example, the first multistep flow-through preparation of a natural product is shown in Figure 3[6]. The synthetic approach is the same as that reported in Figure 1 and it is worth noting the combination between flow chemistry and PASP, as reagents are pumped through various columns packed with immobilized reagents or catalysts. No product isolation at intermediate stages or distillation, crystallization or column chromatography were necessary and the final product was obtained with a purity greater than 90% and in an overall yield higher than 40%. This was accomplished by directly coupling glass reaction columns in series, thereby providing multiple synthetic transformations in a one flow-through process.
Conclusion
The pharmaceutical industry is facing many challenges. The euphoria of the 1990s that resulted from technologies such as HTS and combinatorial chemistry, but also from outstanding scientific progresses, such as the sequencing of the human genome, did not lead to the expected results. In the 1990s, the drug-discovery process focused more on the concept of ‘quantity-based productivity’ rather than on ‘quality-based productivity’, in the hope that statistically this could lead to more new drugs. More biological targets (originating from molecular biology progresses), more screenings (HTS) and larger compound libraries (combinatorial chemistry) indeed produced more lead compounds, but unfortunately the number of new drugs did not increase because the lead compounds identified did not translate into development candidates and, eventually, new drugs.
The pharmaceutical industry is now changing its approach to drug discovery and quality is becoming the new focus. Molecular target validation is increasingly important and the overall quality required for a compound to enter the development phases is higher. The new technologies mentioned in this article, particularly if integrated together and combined to other novel approaches (e.g., fragment-based drug discovery), are valuable tools for the medicinal chemist to remain competitive in this new and highly challenging environment.
Financial & competing interests disclosure
The authors have no relevant affiliations or financial involvement with any organization or entity with a financial interest in or financial conflict with the subject matter or materials discussed in the manuscript. This includes employment, consultancies, honoraria, stock ownership or options, expert testimony, grants or patents received or pending, or royalties.
No writing assistance was utilized in the production of this manuscript.
Bibliography
- 1 Ley SV, Schucht O, Thomas AW, Murray PJ. Synthesis of the alkaloids (±)-oxamaritidine and (±)-epimaritidine using an orchestrated multi-step sequence of polymer supported reagents. J. Chem. Soc. Perkin Trans.1,1251–1252 (1999).
- 2 Gedye R, Smith F, Westaway K et al. The use of microwave ovens for rapid organic synthesis. Tetrahedron Lett.27(3),279–282 (1986).
- 3 Giguere RJ, Bray TL, Duncan SM, Majetich G. Application of commercial microwave ovens to organic synthesis. Tetrahedron Lett.27(3),4945–4948 (1986).
- 4 Giardina GAM, Martinelli M, Mortoni A, Regalia N, Gagliardi S. Microwave-assisted highthroughput chemistry in lead generation and optimization. Presented at: Austrian-German–Hungarian–Italian–Polish–Spanish Joint Meeting on Medicinal Chemistry – JMMC. Vienna, Austria, 20–23 June 2005.
- 5 Breslauer DN, Lee PJ, Lee LP. Microfluidics-based systems biology. Mol. BioSyst.2,97–112 (2006).
- 6 Baxendale IR, Deeley J, Griffiths-Jones CM, Ley SV, Saaby S, Tranmer GK. A flow process for the multi-step synthesis of the alkaloid natural product oxamaritidine: a new paradigm for molecular assembly. Chem. Comm.2566–2568 (2006).